
Process Development 101: How Not to Burn Down Your Factory
Or... how to manufacture your API in the right quantity, at the right cost, and in the right time frame
Scaling-up chemical processes for the manufacture of small molecule pharmaceuticals is not simply about using a bigger reaction flask. Processes that can easily be accomplished at small scale in the laboratory are often not possible at large scale in factories without significant modification. And that’s why process development is key. Process development serves two key functions. Firstly, to manufacture increasing quantities of the API – perhaps scaling from grams to tons over time – to support ongoing clinical testing of the experimental drug, and to support other development activities, such as formulation. Secondly, process development helps to improve the “recipe” to achieve these increasing scales of manufacture in a timely, safe and economic manner – all without polluting the environment, injuring anyone, or burning the factory down! Process development must consider chemical and physical safety; commercial concerns, such as time, cost, and availability of starting materials; chemical, analytical and engineering aspects; and legal and regulatory requirements.
Chemical and physical considerations
The initial chemical route for any new API is likely to have been designed for the facile synthesis of many closely related structural analogues, which can be achieved by relying on late-stage functionalization, as shown schematically in Figure 1 (especially Step 6). For larger quantities, however, the initial route may no longer be viable for a number of reasons. One common problem is that the initial starting material (A) may not be commercially available in sufficient quantities, necessitating additional steps from an earlier starting material (X) and adding expense. In another example, the hazards present in the initial route may be unsafe at a larger scale. The first priority, therefore, is to establish the commercial viability and operational safety of the chosen route. If this cannot be guaranteed, then another route will be required.
Even without these issues, it is likely that a shorter route using alternative, cheaper, and more readily available starting materials (bottom route of Figure 1) to produce the desired analogue will be more economical in the long term. At an early stage, it is beneficial to investigate and patent any other viable routes, even if not used (since it may also hinder competition in the long-term). Route selection is always a key priority.

Figure 1: Schematic of route comparisons for original generic discovery route to prepare multiple analogues (top), and shorter bespoke scale-up route for a chosen product (bottom).
After route selection comes chemical selection. Reagents need to be chosen for suitability, availability, safety, toxicity and expense. Costs that might be tolerated at small scale, as well as toxicity that can be contained within specialist laboratory equipment, may become unacceptable on larger scales. Chemical hazards can arise if the reaction generates excessive heat, for example, which can cause the reaction solvent to boil, potentially causing the vessel to rupture. Likewise, rapid production of gaseous by-products can burst reaction vessels. These and other factors may make some routes, reagents or laboratory techniques unviable for large-scale manufacture, so alternatives must be found.
In terms of physical conditions, the most important factor affecting scale-up results from the square-cube law, which explains how the surface area is inversely proportional to volume (SA/V) (Table 1). For reaction vessels of a similar shape, a 1000 L vessel has proportionately a 10-fold lower SA/V ratio than a 1 L vessel (Figure 2). This affects the rate at which heat can be added and, more importantly, removed from a reaction vessel – for example, during an exothermic reaction. Many chemical reactions generate heat as they progress and proceed faster at higher temperatures. Once heat is generated faster than it can be removed, a “runaway reaction” is inevitable – often with disastrous consequences! For this reason, scale-up is typically limited to 10-fold increments and careful measurement by chemical engineers of the heat produced (reaction calorimetry) is undertaken as scale increases to prevent this.
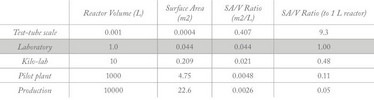
Table 1: Comparison of surface area : volume (SA/V) ratio with reactor vessel scale.

Figure 2: 1 L reactor vessel.
Physical stirring also changes on scale-up. Magnetic stirrers work well in small reaction flasks, but are ineffective above the 1 L scale (Figure 2). Furthermore, much less solvent is typically used in scale-up reactions to increase throughput in plant-scale reaction vessels. This reduces processing times and costs for operations such as heating, cooling and distillation. However, concentrated processes are more likely to be heterogeneous (i.e., contain undissolved solids and liquid together), and even homogeneous reactions may be highly viscous.
Mechanical stirring must be used instead of magnetic stirring, but even so, it may not be fully effective as the scale increases. Different shapes of stirring blades may be needed on a larger scale, and baffles (flow-directing or obstructing panels) may also be introduced into the vessel to increase mixing, especially to lift heavy solids off the bottom of the reactor. It is difficult to predict and model the effects of stirring heterogeneous reactions on scale-up, but it’s wise to be aware of the potential issues from an early stage. Collaboration with chemical engineers is strongly recommended to avoid potential problems.
Choose your solvent
After route and reagent comes solvent selection, although in practice all three are interdependent and investigated alongside one another. The solvent will be the largest single component in any reaction and its selection raises possibilities and challenges. Only a limited number of solvents are cheaply available on a large scale ( and are mostly petrochemical derived). Environmental concerns and increasing regulation mean that fewer solvents are available for use, and restrictions are tightening all of the time. Many chlorinated solvents are now banned at full manufacturing scale, so even if laboratory studies are possible, there is little value in investigating them if their use is prohibited in large scale operations. Early identification of a preferred long-term solvent should be a priority.
Beyond expense and availability, solvents should dissolve substances to reduce the reaction volume needed and help minimize heterogeneity. The properties of the ideal solvent should fall in a “Goldilocks zone”: it should dissolve reactants, but not the desired product so much that crystallizations become difficult. Low-boiling solvents limit the thermal range available for heating, which may slow the reaction rate (and time is money on a large scale!) and reduce the safety margin before boiling in the event of a thermal runaway. Such solvents often also have low flash points, and may generate static when pumped through glass-lined reaction vessels and industry-standard piping.
Conversely, high-boiling solvents offer a wider operating and safety window, but can be difficult to remove from desired products. Such solvents are often water miscible (allowing them to mix in all proportions), which can be advantageous. However, they can also have higher toxicities and costs, making them less desirable. Inevitably, solvent choice is a compromise between multiple factors.

Purifying the product
Lastly, for each step in the selected route, the intermediate product must be isolated and purified on a large scale. For small-scale reactions, intermediates are typically purified by column chromatography. However, this powerful and ubiquitous laboratory technique is disproportionately difficult and expensive to conduct on a large scale – and almost always avoided!
Crystallization is the preferred method for isolation of solid products on a large scale, for which a judicious choice of the solvent plays a part; in fact, the requirements of crystallization are more likely to have determined solvent choice over the reaction. Ideally, the desired product will crystallize directly from the reaction solvent at the end of the reaction, but this is rare. More commonly, another purification will first be required, typically involving additional operations such as extractions and washes. This will likely be followed by distillation, reducing the solvent volume before the more demanding crystallization can be attempted. Consequently, over half the time spent on optimizing a chemical reaction will typically be used to devise a robust and efficient isolation and purification process, rather than on the reaction itself.
Other forms of isolation are also possible. For high-boiling water-miscible solvents, an aqueous drown-out often works well. This involves adding a large volume of water to the reaction mixture, which precipitates the organic product and washes away the solvent and inorganic by-products from the process. However, this technique is inadvisable for late-stage intermediates in the route. It may precipitate all organic components in the reaction mixture, including structurally related organic impurities. Such impurities similar to the API could exhibit deleterious biological activity and so must be controlled to very low levels to protect the patient.
Analytical chemists help monitor and track impurities through the various steps across the project’s lifetime, with increasing scrutiny of impurities at lower levels closer to the API. The process development chemist’s challenge is to achieve increasing purification without recourse to all-powerful chromatography. Indeed, during the initial stages, the API should not be too pure, but have some typical impurities at moderate levels. If these batches are tested and proven clinically safe, any improved processes that follow with lower impurity levels should also be safe. However, the route, reagents and solvent should ideally be fixed before this, since even minor variations in processes can result in significant changes when considering impurities at <0.1 percent in the API.
As the drug candidate proceeds through development, the focus gradually changes from chemistry in the early stages, to engineering in the mid-phase, to analytical in the later stages. Safety remains paramount throughout, firstly for the researchers and operators involved, but ultimately for the patients receiving the new medicine. Commercial and environmental considerations increase in significance throughout the project’s lifetime and it is both the challenge and responsibility of process development to ultimately deliver economically and environmentally sustainable chemical processes for manufacture, which are also high quality, safe and practicable to operate.
Recommended Reading
N. G. Anderson, “Practical Process Research & Development,” 2nd Edition. Referencing press: 2012.
M. Butters et al., “Critical Assessment of Pharmaceutical Processes – A Rationale for Changing the Synthetic Route,” Chem. Rev., 106, 3002-3027 (2006).